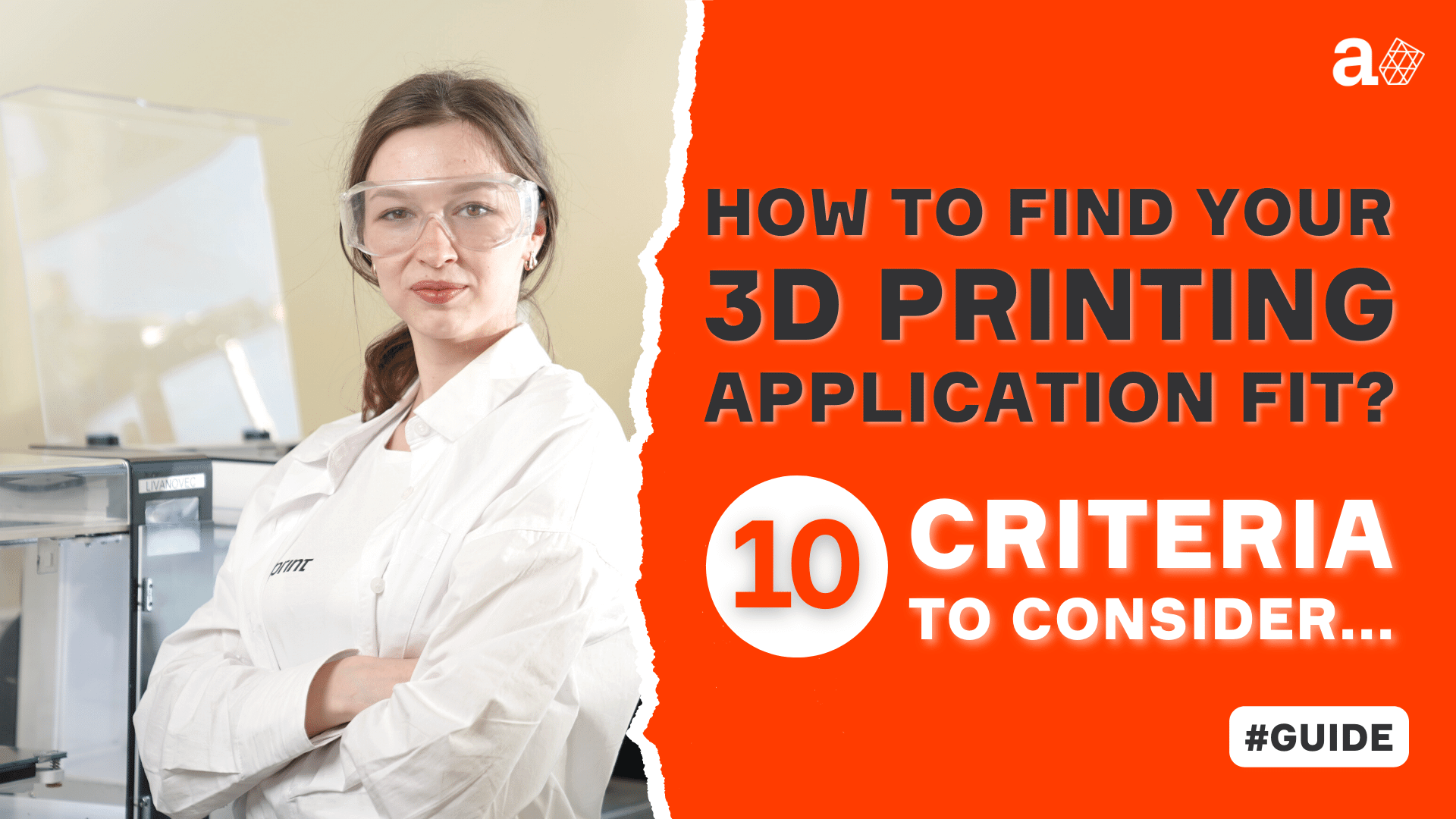
From productivity, to material properties, tolerances and much more – we’re looking at the potentials and the restrictions. And we hope this list can help you to harness the power of innovation and turn it into your unfair advantage that makes manufacturing more sustainable together with saving time and money.
Content
1. Productivity is always a compromise of quality and tolerance.
2. Manufacturing on demand offers immediate advantages of 3D printing.
5. Materials offer a wide range properties, so consider your trade offs and choose wisely.
6. Tolerance, surface finish, and post processing affect costs equally.
7. Service and maintenance is a must for business continuity.
8. Open or closed system? Consumables availability and price make big difference.
9. Don’t underestimate your infrastructure requirements.
10. Ease of use is important for your team to master the new technology.
1. Productivity is always a compromise of quality and tolerance.
Most 3D printing technologies have low productivity and are not suitable for mass production. There is a well-known batch size/cost chart comparing 3D printing vs traditional manufacturing for mass production:
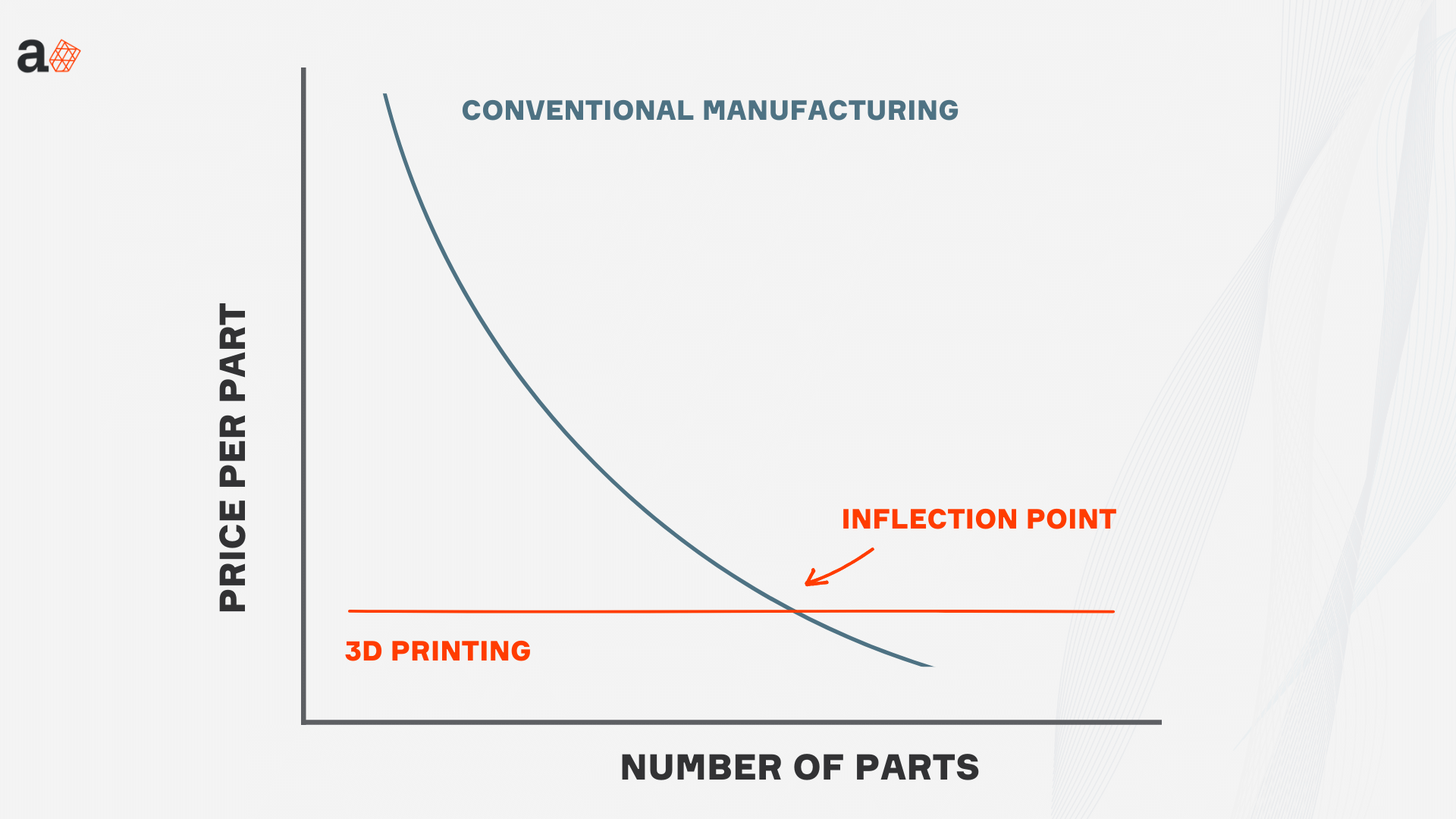
- In 3D printing, the price per part does not depend on the quantity of parts made.
- For most traditional manufacturing processes it is exponentially decreasing.
- For each particular comparison case (application, technologies in comparison) there is a certain intersection point: If the quantity is lower than at the intersection, it becomes profitable. For larger quantities – traditional manufacturing is more suitable. On average, this number is around 1000-5000 parts per year.
Productivity is one of the biggest barriers preventing AM implementation in production at scale. It can change only if the manufacturing paradigm shifts from centralized manufacturing (giga factories) to localized manufacturing (microfactories), like for Local Motors or Arrival concepts.
Challenging the traditional production line approach, Microfactories allow the process to be more dynamic and flexible, with each robotic cell having multiple functions rather than just one sole purpose. Arrival’s Microfactory approach not only sets out to revolutionise the manufacturing of electric vehicles but also has the potential to become a new norm for factory production.
Productivity is expressed in mass per time or volume per time. Typical FDM systems have productivity of 10-20 grams per hour. There are systems with higher productivity such as Essentium, which uses high speed motion kinematics or Thermwood that uses pellets and very big nozzles. It can be tuned by adjusting print speed or nozzle size, but it is always a compromise with quality and tolerance.
Typical print time for an FDM part of 100-200 mm diameter is 1-2 days, and depends on part geometry, possibility of using grid infills, strength and stiffness requirements, supports etc.
2. Manufacturing on demand offers immediate advantages of 3D printing.
This topic is connected to productivity. Currently, most of the existing proven 3d printing applications concentrate on low volume or individual parts production. For certain f applications mass production is not required or possible: This is where 3D printing gives a number of immediate advantages. Such applications include:
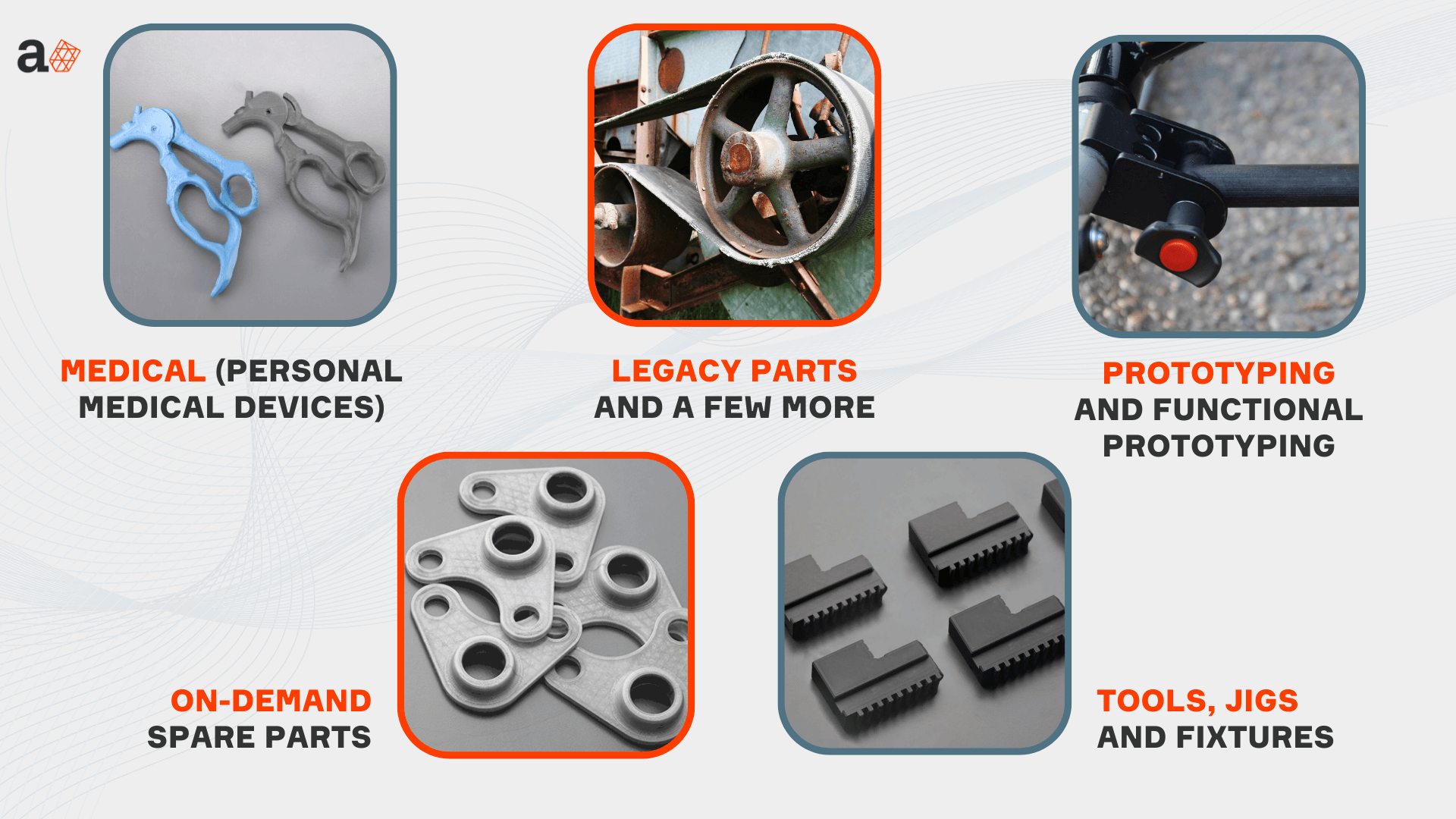
- Medical (personal medical devices),
- prototyping and functional prototyping,
- tools, jigs and fixtures,
- on-demand spare parts,
- legacy parts and a few more.
Important criteria to consider are geometric limitations of the technology (eg tolerance requirements, need of supports, capabilities of manufacturing cavities, thin walls etc). Slicer capabilities and usability is also important when considering a technology or products for on-demand manufacturing.
3. Design for additive manufacturing (DfAM) and slicing improves costs, efficiency and production time of the printed parts.
Most of AM technologies require certain design approaches. This is especially important for end-use parts, but proper designs can significantly reduce cost, production time and efficiency of 3D printed parts. Many parts that are not designed for AM behave poorly or unpredictably when printed without considering design changes or tuning of process parameters.
Here, two important criteria are the availability of tools (CAE/CAM) and their capabilities, as well as educational materials, training courses and other technology knowledge. Different OEMs offer different CAE/CAM (slicer) software. Each has different capabilities, interface, options, price, etc.
At Anisoprint, we developed our own proprietary software for slicing: Aura. It allows to create design for anisotropic structures and enhance internal structure of parts with custom multimaterial areas. There is also a comprehensive educational course for mastering 3D printing anisotropic composites.
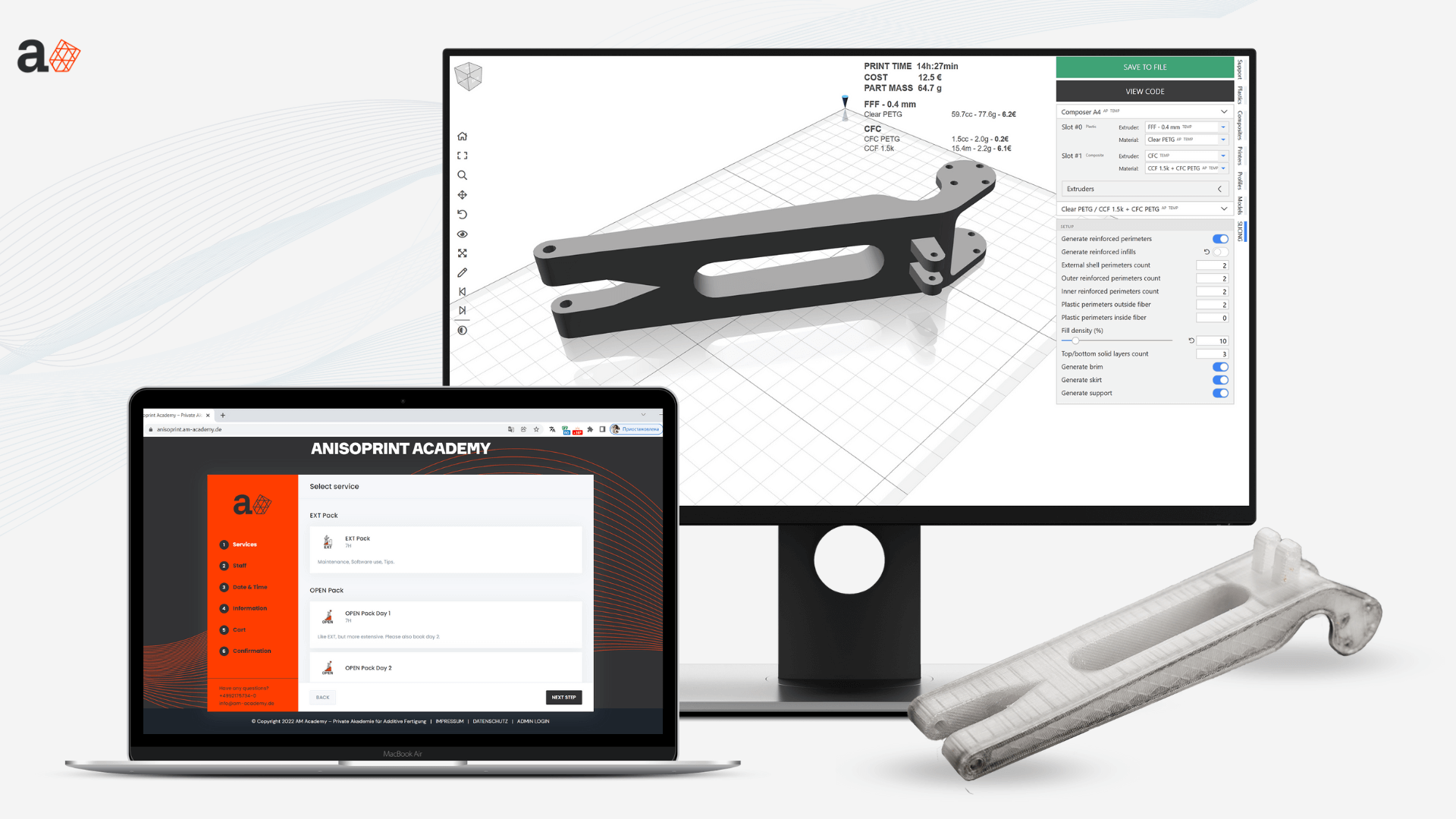
4. Certification is a purely administrative barrier but mandatory for most parts, materials and processes.
For functional usage, many parts (especially end-use parts), materials and processes require certification. This is extremely important for life-critical components and primary structures of machines and devices. Most prototyping or tooling applications do not require mandatory certification, but some still do. This depends on industry, country, use cases and it is normally the topic that the end user is much more aware of compared to OEM.
The majority of AM technologies and materials are not certified. There are times when the certification process is fast, but there are also times when it takes decades and tens of millions (such as primary structures of passenger airplanes). Machines themselves might also require certification, from simple electric equipment certification (CE in EU or UL in US) to more complex industrial equipment certification. This topic is mostly resolvable by joint effort of OEM and end user in several months, as it is a purely administrative barrier, not a technology barrier.
5. Materials offer a wide range properties, so consider your trade-offs and choose wisely.
This is one of the most broad topics. What to consider here:
5.1. Mechanical properties.
5.1.1. Strength
Different technologies use different materials and strength can vary significantly, here are some examples:
Material | Strength, Mpa |
---|---|
Low-end resins for SLA | 5-10 |
Engineering thermoplastics | 80-100 |
Aluminum alloys | 200-400 |
High strength steels | 1000-1500 |
Composites | 200-1500 |
Strength is important in use cases with clear loading conditions (known forces/pressure applied) and where these forces are rather high.
It’s also important to distinguish between strength and structure. Consider your trade offs and choose wisely: Increasing thickness will increase strength, weight, material usage, and print time. It is a very important parameter to optimize costs and production time. There are applications with unknown or rather unpredictable loads, so strength plays an important role there. A plastic part may break under certain conditions like shocks or wear, so metals or composites are preferred choices.
5.1.2. Density, or weight. Important for lightweight components: for aerospace, automotive, medical applications, handled parts, etc. They are easier to handle, process and recycle.
Most plastics have density in the range of 1-1.5g/cm3, fiber reinforced polymers are slightly heavier (1.2-2.5g/cm3), and metals are obviously the heaviest (from 2.7 for aluminum to 7.8 for steel).
Stronger materials allow manufacturing more lightweight parts due lower material volume needed to withstand the same loads. That’s why it makes sense to consider strength and density together by introducing relative, or specific strength – strength to weight ratio.
Polymers have lowest specific strength among other structural materials used in AM, metals are several times better: e.g. stainless steel is 7 times heavier but 20 times stronger than ABS, it makes the specific strength 3 times higher.
Carbon fiber reinforced polymers (CFRPs) – but only the ones with continuous or long fibers – have the highest specific strength amongst all structural materials, up to 10 times higher than metals. Chopped fiber filled polymers have the more or less the same specific strength as the ones that are not reinforced.
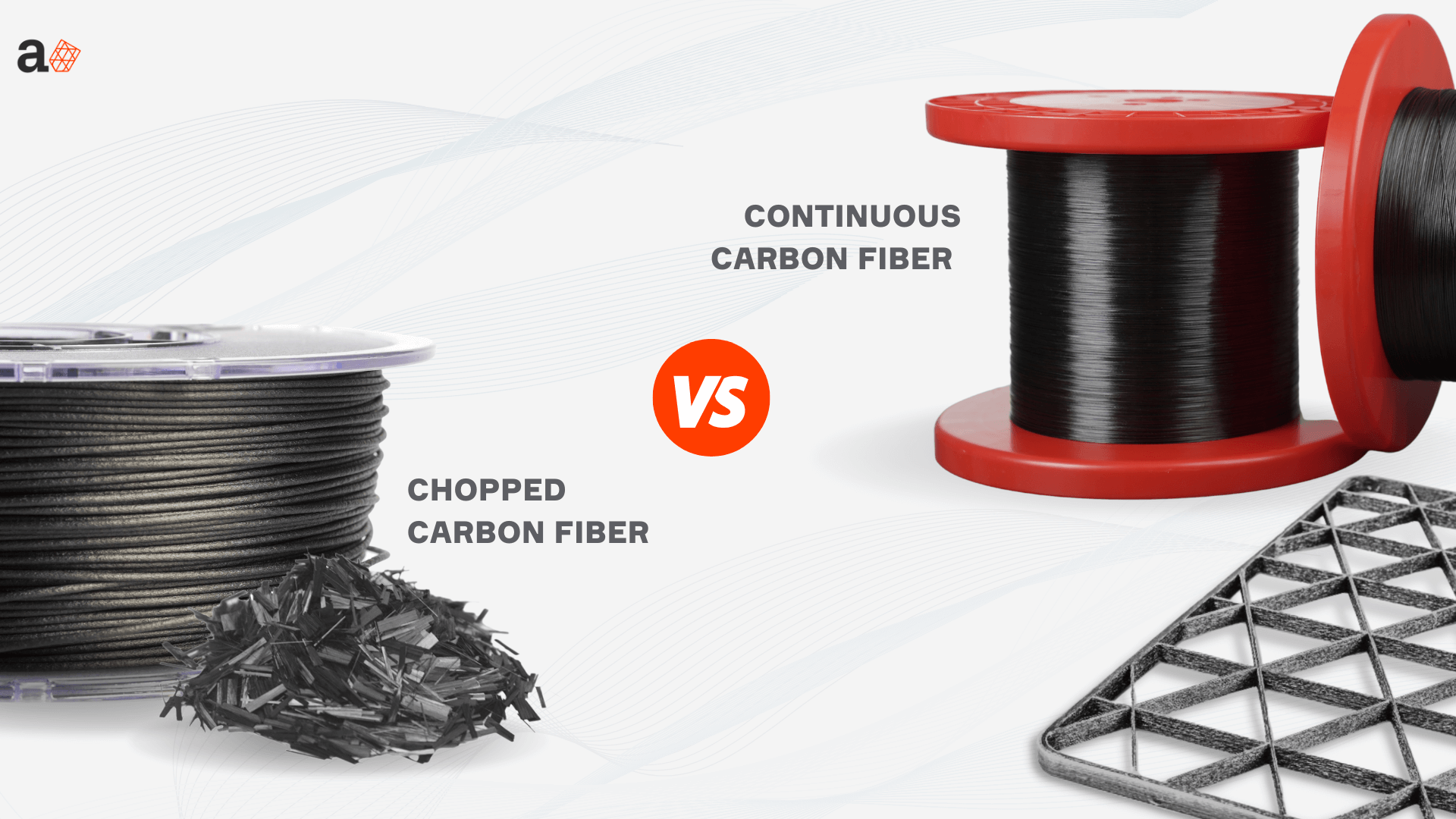
5.1.3. Stiffness. Stiffness is another important material property. It can also be either material (Young’s modulus) or structural (deformability). Stiffness is much more dependent on the structure (geometry, inertial properties) rather than on material itself.
Typical elastic modulus: |
---|
for polymers is around 1-3 GPa, |
for aluminum – 70 GPa, |
for steel – 210 GPa, |
for CFRPs – 60-140 GPa (depending on the fiber volume fraction). |
Short fiber filled polymers normally have 1.5-2 times higher elastic modulus compared to unreinforced. Thus, an aluminum beam will have the same stiffness as a 3 times thicker plastic beam. Stiffness is important for tolerance under loads (e.g. fixtures for milling or pressure processing). It also affects buckling failure modes.
5.1.4. Surface toughness. Surface toughness is important for indentation or wear applications. Soft surfaces wear out faster in contact applications (e.g. gears or friction pairs). Plastics have low surface toughness compared to metals and ceramics. As it is a surface property, CFRPs normally have plastic on the surface, so toughness is similar to polymers. Chopped fibers can slightly increase it.
5.1.5. Frictional properties. There are certain types of polymers that have a very low friction (so called self-lubricating polymers). Such polymers can be used efficiently in contact (friction or wear) applications, with no need of grease.
5.1.6. Plasticity, or elongation at break. Most materials exhibit permanent, or plastic, deformation, under high loads. Under plastic deformation, material begins to “flow”, and can deform significantly before it actually breaks.
Plastics, obviously, can have fairly large plastic deformations before failure, in hundreds of percent. Metals can flow up to 10-20%. Resins and ceramics are brittle. There is a practical rule that stronger materials are normally less ductile (more brittle) – they have lower elongation at break. For high strength maraging steels it can be just 1-2%. Classical epoxy-based CFRPs are even more brittle.
This characteristic is extremely important for shock or impact loadings. Material can have a very high strength, but it can break easily under so-call “hard” loading regimes such as shocks.
Adding short fibers to polymers significantly decreases their plasticity, they become more brittle, but can still absorb shocks well due to other effects. This characteristic also affects failure modes/failure surfaces. Brittle materials will crack with high energy release, plastic materials will flow.
5.2. Thermal properties.
Materials change their behavior when the temperature changes. As a rule, with the increase of temperature, strength and stiffness decrease. At low temperatures, stiffness increases, but the material can become very brittle. At certain temperatures, materials will start to flow, burn or degrade. It defines the operational temperature range.Heat conductivity is another important thermal property. Typically, metals are good heat conductors, so they are good for heat sink or heat transfer applications. Polymers normally are not that thermal conductive, so are better for thermal insulation.
For most polymers, it is between -50 and + 100°C. There are high temperature polymers that can withstand up to 200°C and more, and low temperature polymers that will still behave reasonably well at cryogenic temperatures. Metals are normally more temperature resistant. Aluminum can work up to 200-300°C, steel up to 400-500°C, Nickel alloys up to 800°C, ceramics up 1000°C and more. CFRPs are slightly more temperature resistant compared to polymers.
Heat conductivity is another important thermal property. Typically, metals are good heat conductors, so they are good for heat sink or heat transfer applications. Polymers normally are not that thermal conductive, so are better for thermal insulation.
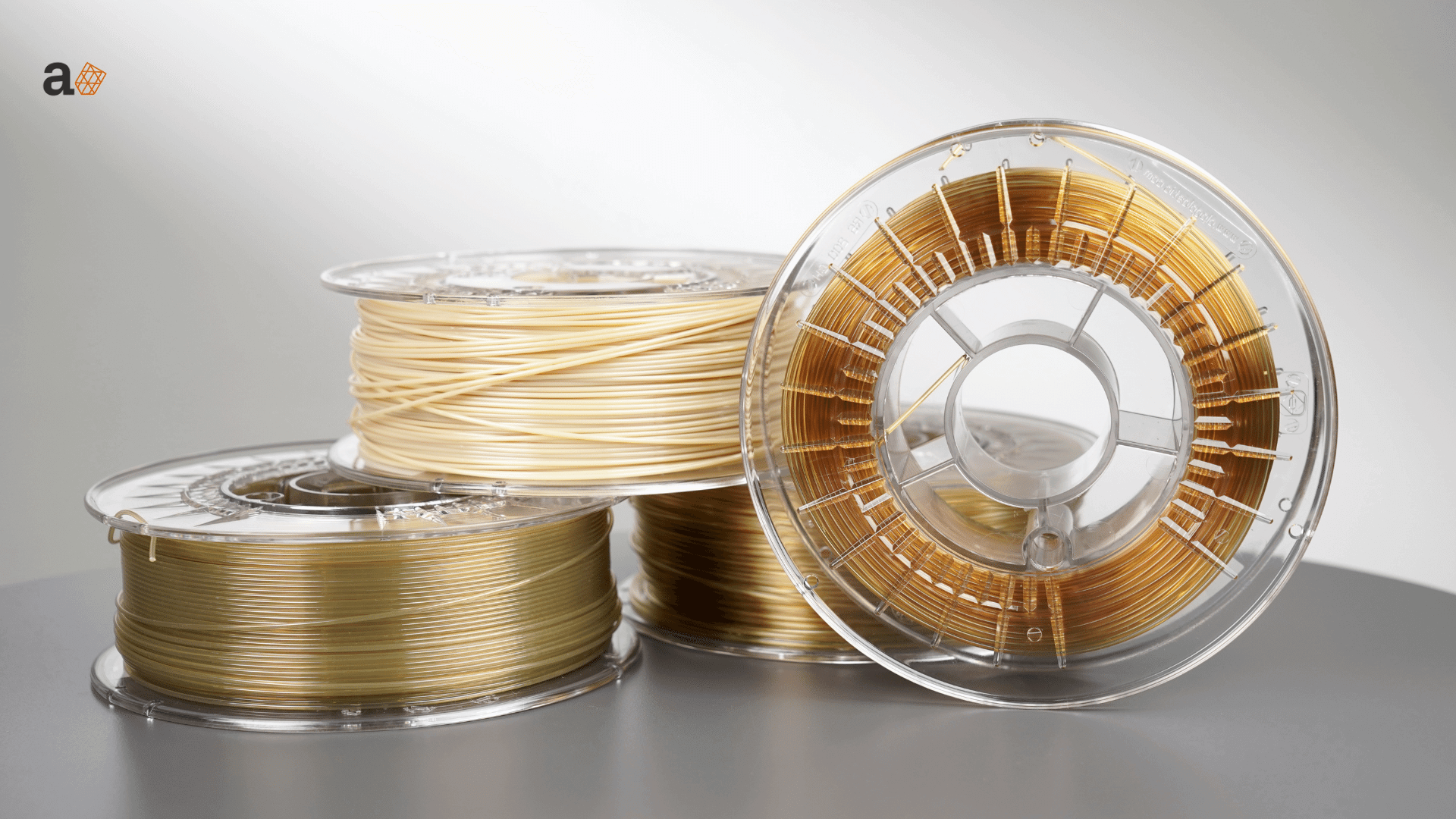
5.3. Physical properties.
Physical properties include resistance to light, moisture, radiation, corrosion, fungi, and many more. Physical properties may vary depending on the application. Polymers offer the widest range of physical properties for different applications.
5.4. Chemical properties.
These properties include chemical resistance to certain media, such as solvents, fuels, lubricants, acids, etc, as well as adhesive properties, which are the strength of bonds they form. Depending on their chemical resistance and adhesion properties, polymers can offer a wide range of options.
Another important property is flame retardancy. The majority of polymers burn and sustain fire, which is problematic for some applications. But there are “V0” polymers as well
5.5. Electrical and magnetic properties.
Materials can either conduct current or they don’t, this is pretty obvious. Most metals are conductive, polymers are not.
Carbon fibers are conductive, so adding them to polymers can create a conductive compound.
Electrostatic properties are also important. Many applications require electrostatic discharge. Certain polymers can have that. Conductivity also influences radio transparency.
Glass fibers are not conductive and radio transparent.
It is important to consider if the technology or machine can process materials that meet the requirements of your applications. If the list of applications and use cases is wide and open, it is better to consider open material systems that allow to use different third-party materials and not limit the end user to only OEM materials. Open systems normally require a strong background in composites, more skill to use, but applications are not limited.
5.6. Processability, technological properties.
This includes if the material or technology requires certain handling or preprocessing of raw materials. For example, most polymers absorb moisture. High moisture content will affect print quality, so materials need to be dried.
6. Tolerance, surface finish, and post processing affect costs equally.
For tolerance it is rather simple: each machine, material, and process has a certain tolerance capability.
Surface finish and post processing is a more complex topic. There is almost always visible layer structure on the side surface of the print. Layers might have different thickness, which would make them more or less visible.
SLA or SLS layers could be as thin as 10-50 micron. For FDM technology, surface finish also depends on layer thickness (thickness tolerance) and material properties. For FDM you can get quite a large variation of layer thickness options, from 10 micron to several millimeters or even centimeters.
Thin layers will not only improve surface finish, but will significantly decrease productivity. Big FDM machines such as Thermwood can print with 10mm nozzles and 6-10mm layers with up to 200kg/hr. Certain high-tolerance FDM machines can print very thin layers with a 0.1 mm nozzle, but the productivity will go down to 1 gram per hour.
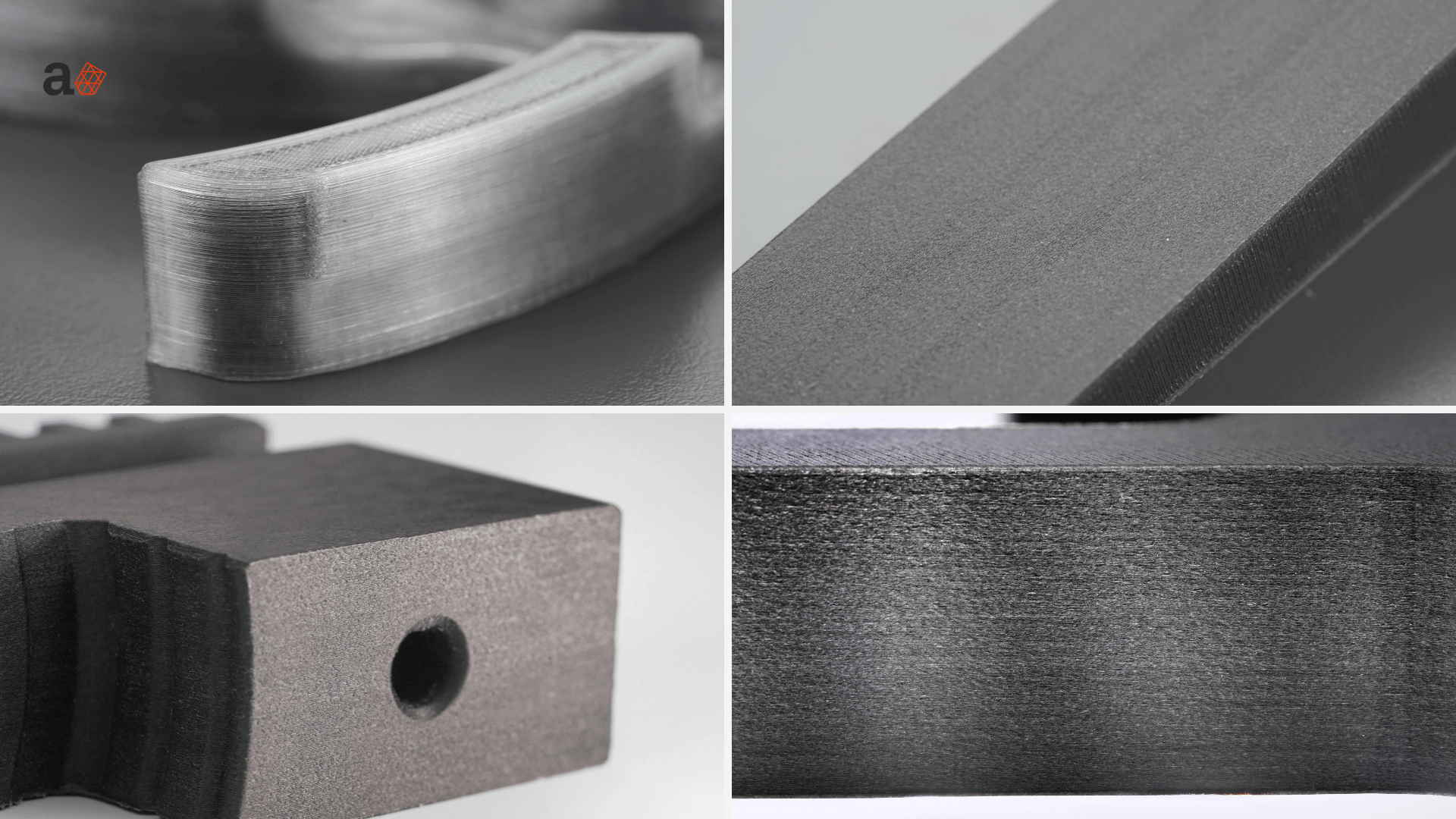
If surface finish is important, post processing is possible. There are dozens of post processing techniques available for different AM technologies, like sanding, tumbling, cutting, coating, chemical smoothing, etc.
7. Service and maintenance is a must for business continuity.
This topic is strongly connected to system reliability and the MTBF – mean time between failures. Most AM systems are still rather new, so reliability is sometimes an issue. To avoid mean times, timely service and spare parts availability is extremely important, as well as qualification and proximity of service technicians and responsiveness of customer support.
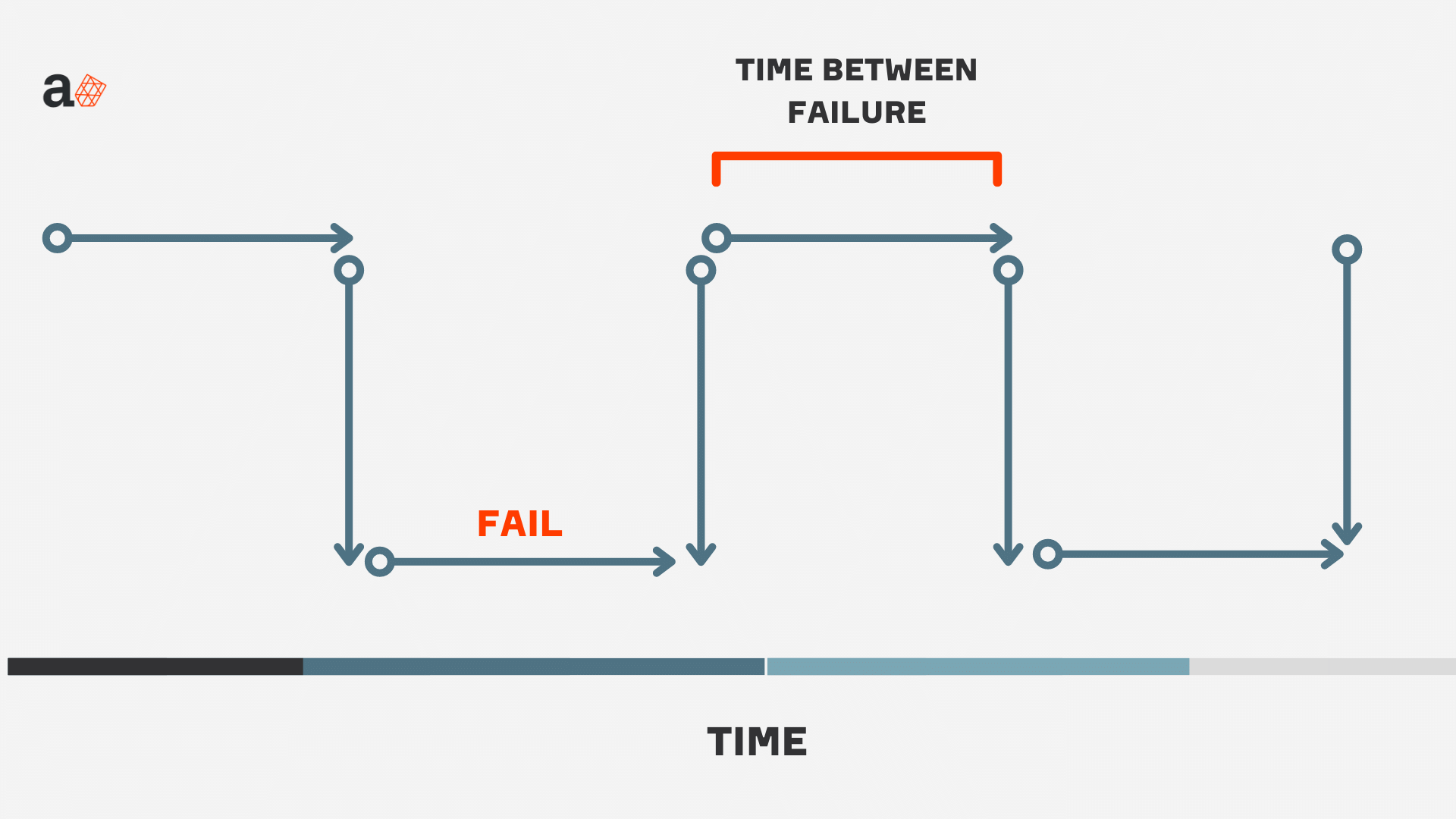
8. Open or closed system? Consumables availability and price make big difference.
There are closed systems that only allow the use of OEM-provided materials – Stratasys, Desktop Metal, Markforged, etc. Closed systems can ensure high quality and repeatability, but the material options are limited, prices higher than market average and supply chain risks are the drawbacks.
Open systems, such as Ultimaker, Raise 3D or Anisoprint, leave much more options to the user in terms of available materials: there is always a choice between competitive options so the price is lower, and the supply chain can be diversified. But each new material will require significant effort from end-users to tune Aura settings for them.
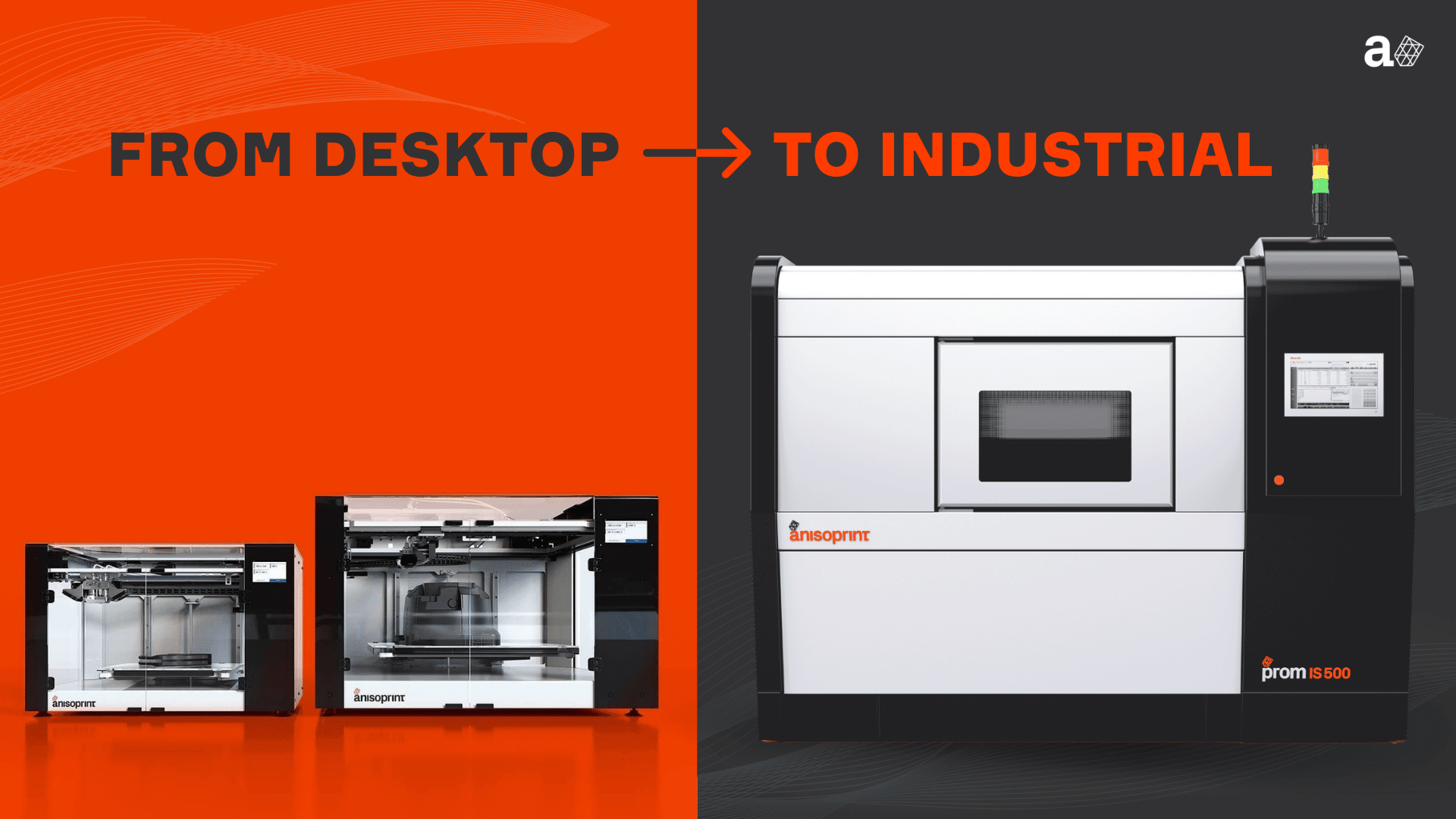
9. Don’t underestimate your infrastructure requirements.
Each machine and technology has different requirements for the end-user infrastructure. Some systems are low-energy, desktop and even office-friendly. Some require special gasses, material handling etc. Think of one that fits the facility.
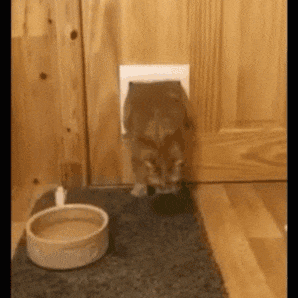
10. Ease of use is important for your team to master the new technology.
3D printers are generally more user friendly compared to traditional manufacturing machinery, though they still require certain education and skills. FDM is, by the way, contrary to the hardware price and complexity, one of the most hard-to-operate and hard-to-master technologies among all additive manufacturing technologies. So it is important to pay attention to the level of automation, sensors, safety, self service and other parameters.
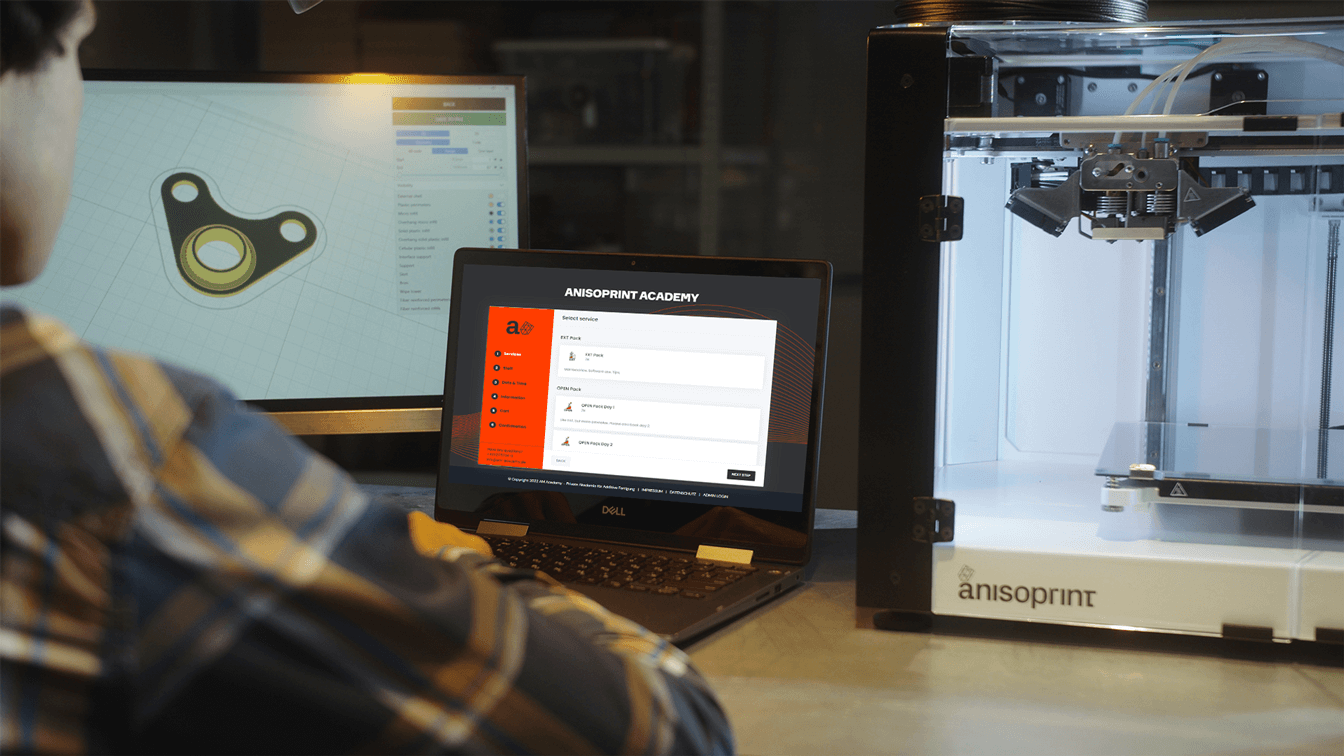
That’s it! You have some unanswered questions? Fill in the form below and we’ll get back to you!
Reference
MJF – Multi Jet Fusion (MJF) is a powder-bed 3D printing process developed by HP that bonds agent and powder in a process similar to binder jetting. Unlike point-to-point laser-based powder-bed fusion systems, MJF selectively distributes fusing and detailing agents across a bed of powder, and layers are fused together using infrared light1.
BJ – The binder jetting 3D printing technique consists of the deposition of a binding adhesive agent onto thin layers of powdered material. The powdered materials are either ceramic-based (for example glass or gypsum) or metal (for example stainless steel)2.
FDM – Fused deposition modeling (FDM), also known as the material extrusion additive manufacturing technique, utilizes polymers as the raw material (filament). The filament is usually heated to a molten state and then extruded through the nozzle of the machine (3D printer)3.
SLS – Selective laser sintering (SLS) is an additive manufacturing (AM) technique that uses a laser as the power source to sinter powdered material (typically nylon or polyamide), aiming the laser automatically at points in space defined by a 3D model, binding the material together to create a solid structure. It is similar to selective laser melting.
SLM – Selective laser melting (SLM) is one of many proprietary names[1] for a metal additive manufacturing (AM) technology that uses a bed of powder with a source of heat to create metal parts. Also known as direct metal laser sintering (DMLS), the ASTM standard term is powder bed fusion (PBF).
SLA – Stereolithography (SLA or SL; also known as stereolithography apparatus, optical fabrication, photo-solidification, or resin printing) is a form of 3D printing technology used for creating models, prototypes, patterns, and production parts in a layer by layer fashion using photochemical processes by which light causes chemical monomers and oligomers to cross-link together to form polymers.
DLP – Digital Light Processing is a 3D printing (additive manufacturing) technology which utilizes light and a liquid resin to make solid parts and products4.